Opportunities and challenges for CAR T-cell therapy in cancer
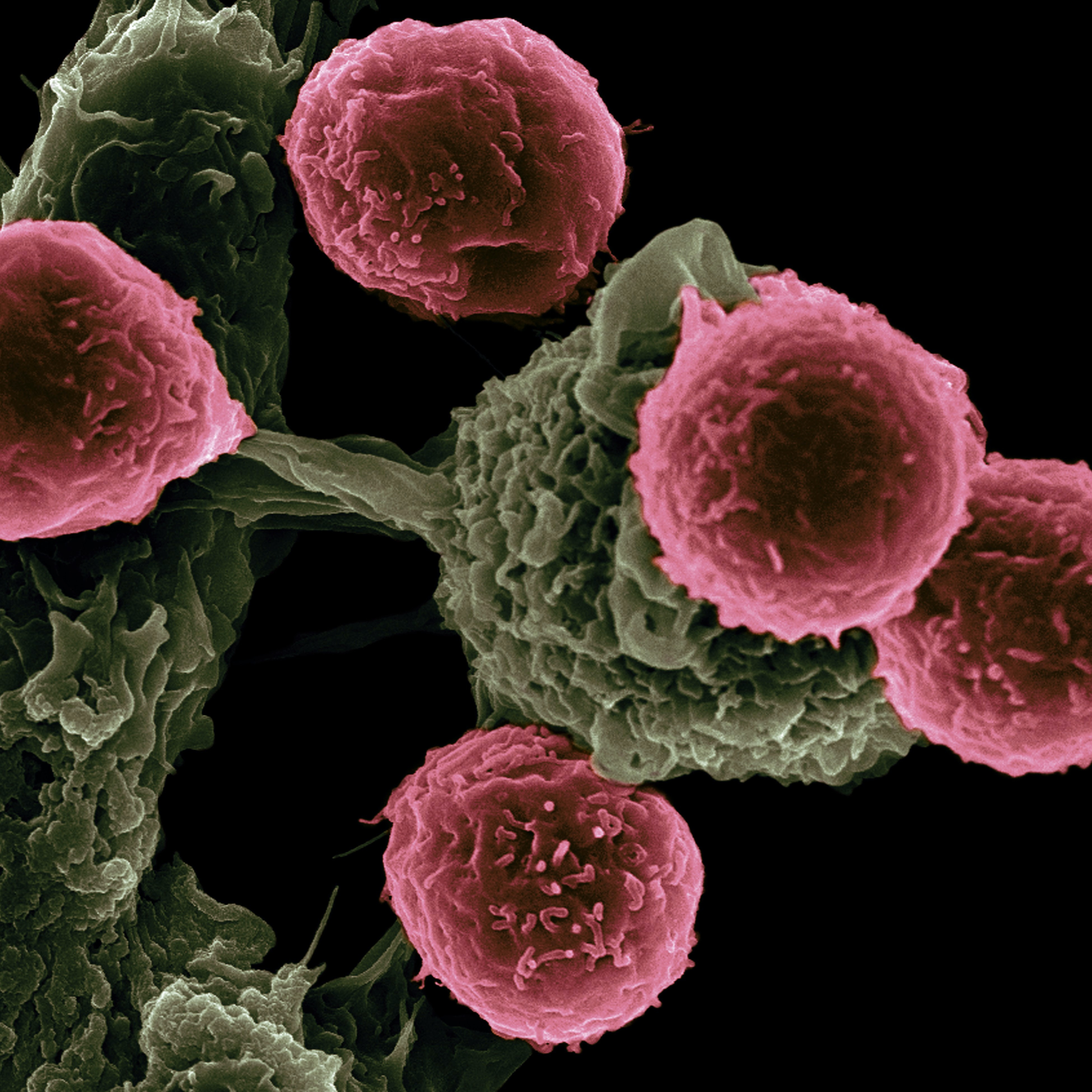
Abstract
Chimeric antigen receptor (CAR) T-cell therapy is a novel therapeutic T-cell engineering option, where T-cells obtained from a patient’s blood are engineered ex vivo to express specific tumour antigen receptors. The highly selective nature of CAR T-cell therapy has led to a revolution in cancer treatment. The use of CAR T-cell therapy has been successful in treating haematological malignancies and there is also a growing interest in using CAR T-cell therapy to target solid tumours. However, there are notable challenges with CAR T-cell therapy, including non-sustained responses, antigen escape, and life-threatening adverse effects. Studies are underway to improve the safety of CAR T-cell therapy by limiting their expression, producing switchable CAR T-cells, and producing genetically engineered T-cells that are equipped with genes to reduce adverse effects.
Introduction
The interaction between the immune system and tumours during the process of disease development and progression to metastasis is a key hallmark of cancer (1). In 1909, Paul Ehrlich proposed that the immune system can recognise and eliminate tumours (2). Subsequently, the idea of an “immunological surveillance mechanism” emerged. In 1959, Lewis Thomas suggested that the human immune system can recognise tumour-specific antigens on the surface of newly developing tumour cells as ‘foreign’ and eliminate them (3). Following this, in 1971, Sir Frank Mac Farlane Burnet hypothesised that tumour cell antigens induced immunological reaction against cancer and postulated the “immune surveillance theory” (4).
Though previous research has established how cancers evade the immune system, much progress is yet to be made in managing cancer by targeting that mechanism. This review includes the biological basis of Chimeric Antigen Receptor (CAR) T-cell therapy as a cancer treatment modality, the opportunities and challenges that CAR T-cell therapy offers, and briefly discusses the role of natural killer (NK) cells in cancer management.
Biological basis of CAR T-cell therapy
The immune system interacts closely with tumour cells via complex mechanisms and can result in either eradication of the tumour or immune evasion by the tumour (5). Generally, CD8+ cytotoxic T-cells and CD4+ helper T-cells, which belong to the adaptive immune system, control the development of cancer via mechanisms like the production of pro-inflammatory cytokines (eg. interferon (IFN)-γ and cytotoxins) (5).Recognition of antigens by T-cells is mediated by T-cell receptor (TCR), which binds to peptides presented by Major Histocompatibility Complex (MHC) found on the surface of antigen-presenting cells (6). After the binding of the MHC/peptide complex to the TCR, the TCR heterodimer interacts with the antigen and facilitates clustering of the CD3 complex, which mediates further signalling that initiates T-cell activation (6).
However, the tumour may evade immune responses through regulatory T-cell-mediated immune suppression in the tumour microenvironment, defective antigen presentation, and production of immunosuppressive cytokines (5). Constant stimulation of T-cells can lead to T-cell exhaustion, loss of T-cell function and reduced ability to proliferate, impaired cytotoxicity as well as decreased cytokine production (7). Consequently, immune cells fail to recognise and kill tumour cells.
In response, CAR T-cells have been designed with receptors that can proliferate and retain their function following antigen exposure (6). CAR T-cells are T-cells that have been engineered to express receptors that specifically target tumour-associated antigens (8). CAR T-cells consist of an antigen-binding extracellular domain derived from a single chain variable segment (ScFv) of an immunoglobulin, a spacer domain, a transmembrane domain and an intracellular domain (8, 9). The intracellular domain of the receptor distinguishes the different CAR T-cell generations as shown in Figure 1.
Figure 1: Generations of CAR T-cells
First-generation CAR T-cells consist only of the TCR-derived CD3ζ intracellular signalling domain, which induces T-cell activation as illustrated in Figure 1 (6, 8). The second-generation CAR T-cells consist of one additional co-stimulatory component (mainly CD28, CD137 or CD134) in the intracellular domain, which amplifies anti-tumour responses in vivo (8). The third-generation CAR T-cells consist of two co-stimulatory components in the intracellular domain (8). Lastly, the fourth-generation CAR T-cells consist of a promoter region that induces cytokine production once CAR T-cell has been activated (8).
Chimeric Antigen Receptor (CAR) T-cell therapy
Figure 2: CAR T-cell therapy methodology
As summarised in Figure 2, CAR T-cell therapy involves the collection of T-cells from the patient by leukapheresis or peripheral blood (9). Following this, T-cells are transduced with specific DNA using viral vectors and allowed to proliferate (9). Subsequently, the CAR T-cells are infused into the patient after the patient undergoes chemotherapy to reduce the number of leukocytes in their blood (9).
Currently, NICE recommends CAR T-cell therapy for patients with refractory diffuse large B‑cell lymphoma and primary mediastinal large B‑cell lymphoma in whom two or more systemic therapies have failed as well as patients aged up to 25 years with B‑cell acute lymphoblastic leukaemia (10, 11). Moreover, caution is advised in patients with autoimmune disorders and solid organ transplants (12).
Opportunities for CAR T-cell therapy in cancer
In the first CAR T-cell trial, CAR T-cell therapy was tested in patients with advanced epithelial ovarian cancer and metastatic renal cell carcinoma, targeting the folate receptor and carbonic anhydrase IX, respectively (13, 14). However, a breakthrough was achieved when CD19-specific CAR T-cells were able to target haematological malignancies effectively.
CAR T-cell therapy offers therapeutic benefits in cancer due to its ability to recognise and bind to antigens expressed on the surface of tumour cells. Unlike the physiological TCRs present in the body, CAR T-cells function independently of the MHC/peptide complex, which helps to overcome resistance mechanisms like downregulation of MHC and defective antigen processing (6, 8). Besides, CAR T-cells only target cell-surface antigens and use alternative ligands to help bind to the target antigen (6, 8). While the extracellular domain of CAR T-cells protects them from tolerance mechanisms, they may induce host responses (8).
CAR T-cell therapy is highly selective. CAR T-cells only interact with cells that express a particular antigen on their surface, which are referred to as tumour-associated antigens (TAAs). In 2017, Wang et al. showed that infusion of variant III of the epidermal growth factor receptor (EGFRvIII), a frequently observed TAA in glioblastoma, was well-tolerated without off-tumour toxicities (15). In 2017, Xia et al. demonstrated that CD19, which is only expressed in mature B-cells, is being used as a target for immunotherapy in B-cell malignancies (16). It has been well-accepted that there is a TCR affinity window, within which TCR with greater affinity has improved antigen recognition (15). Beyond a threshold level of TCR affinity, there is maximal T-cell anti-tumour activity, with no further T-cell activation (15). In such cases, to improve the sensitivity of CAR T-cells, scFv affinity is adjusted to allow greater recognition of antigen targets that are overexpressed on tumour cells (15). Moreover, CAR T-cell therapy has been shown to have broader applications in other haematological malignancies apart from B-cell lymphoma. Similar to targeting CD19 antigen in B-cell malignancies, B-cell maturation antigen (BCMA) is used as the TAA for CAR T-cell therapy in multiple myeloma (17). BCMA, which is present on multiple myeloma cells, belongs to the tumour necrosis factor (TNF) receptor superfamily and is required for the long-term survival of the plasma cells (17). Therefore, the highly-selective binding of CAR T-cells to tumour-specific antigens can modify steps in the progression of cancer.
Apart from haematological malignancies, there is also a growing interest in using CAR T-cell therapy to target solid tumours. Solid tumours refer to malignancies like sarcomas and carcinomas, in which the abnormal mass of tissue does not contain cysts or liquids (16). While haematological malignancies tend to spread via blood, a solid tumour is often a concrete mass that is located at one particular site, especially at an early stage (16). As the target antigens for solid tumours are heterogenous, it can be difficult to design a particular antigen-specific CAR T-cell (16). Currently, CAR T-cell therapy for solid tumours targets key antigens involved in tumourigenesis, including the epidermal growth factor receptor (EGFR), EGFRvIII, human epidermal growth factor receptor 2 (HER2) for breast cancer, carcinoembryonic antigen (CEA), disialoganglioside 2 (GD2) for neuroblastoma, prostate-specific membrane antigen (PSMA) and interleukin-13Ra2 (IL13Ra2) for glioblastoma (18). Pre-clinical studies are underway for the use of CAR T-cells in pancreatic ductal adenocarcinoma (PDAC). In PDAC, the tumour antigen mesothelin, expressed on the cell surface, is targeted (18, 19). Akce et al. showed that mesothelin upregulates tumour growth and invasion (19). Also, localisation of mesothelin to mesothelial cells, with limited expression in normal tissues, enhances the specificity of CAR T-cell therapy (19). Mouse models and in vitro studies using CAR T-cells have achieved significant success in the treatment of solid tumours like breast cancer, lung cancer and colorectal cancer (20, 21, 22). Therefore, despite the heterogeneity of target antigens in solid tumours, key antigens involved in tumourigenesis can be identified and targeted effectively.
CAR T-cell therapy is also able to overcome the adverse tumour microenvironment associated with solid tumours. Particularly, the immunosuppressive tumour microenvironment recruits immunosuppressive cells like myeloid cells and fibroblasts, which form a fibrotic matrix that inhibits infiltration of T-cells into the tumour (16, 23). CAR T-cells can produce immunomodulatory substances, enhance trafficking via expression of chemokine receptors, and convert immunosuppressive tumour microenvironment into one where T-cell activity is enhanced (19). However, the efficiency of CAR T-cell therapy can be reduced by unfavourable tumour microenvironment. Various mechanisms like oxidative stress, acidic pH can threaten the efficiency of CAR T-cell therapy and make them dysfunctional before long (16). In 2016, Wang et al. found that polymorphonuclear myeloid-derived suppressor cells (MDSCs), which are immunosuppressive cells, play a critical role in prostate cancer progression (24). MDSCs secrete chemokines, cytokines, and enzymes, which suppress T-cell activity and prevent CAR T-cell migration to an advanced tumour site, inhibiting the subsequent binding of CAR T-cells to tumour cells (16, 24). Other mechanisms like production of immunosuppressive cytokines and checkpoint inhibitory proteins can reduce the efficacy of CAR T-cells (25). New-generation CAR T-cells are being generated with unique immune-stimulatory actions and have been shown to overcome the immunosuppressive tumour microenvironments in mice or in vitro models (25). Examples include CAR T-cells that constitutively express CD40 ligand, interleukin 8 or PD‐1‐blocking scFvs (25). This highlights the need to design CAR T-cells that can survive the unfavourable tumour microenvironment and are long-lasting.
Novel strategies have also been developed to generate CAR T-cells that can recognise multiple antigens (15). In one method, CAR T-cells are modified to encompass two distinct receptors that bind to two different antigens (15, 26). In 2013, Hegde et al. generated CAR T-cells for glioblastoma that were simultaneously expressing HER2 and IL-13Rα2, both of which were found to have the highest co-prevalence in tumour samples (26). The results of the study illustrated that the use of such genetically engineered CAR T-cells induced more efficient depletion of tumour cells than conventional single-antigen-recognising CAR T-cells (26). This means that the activity of genetically engineered T-cells can continue to persist even if one antigen is downregulated. However, with CAR T-cells that recognise multiple antigens, it is possible to have a disproportionate expansion of one CAR T-cell population compared to another (27). As such, in another method, one CAR T-cell is designed to bind to more than one antigen (15, 26). For example, CD19-OR-CD20 CAR T-cells were developed and were found to control both wild-type and CD19− mutant B-cell lymphomas with equal efficacy (27). Therefore, novel strategies have been developed to circumvent resistance mechanisms.
To improve the safety of CAR T-cell therapy, studies are being done to limit CAR T-cell expression, produce switchable CAR T-cells and produce genetically engineered T-cells that are either equipped with suicide genes or include synthetic control devices that help reduce adverse effects when used in patients (14, 15). Retroviruses and lentiviruses are most frequently used as viral vectors in CAR T-cell production (15). These two vectors have low intrinsic immunogenicity and integrate the transgene molecule coding for CAR T-cell expression at specific loci, reducing potential insertional mutagenesis (28). Furthermore, the switchable CAR T-cell design requires the formation of a receptor complex in the presence of switch molecules that serve to connect domains of the CAR structure or enhance interaction between the tumour antigen and the CAR T-cells (15). In 2018, Raj et al. noted that the administration of switchable CAR T-cells followed by Fab-based switch directed against HER2 showed high efficacy against advanced pancreatic tumours (29). As the dose is titratable, the switchable CAR T-cell therapy can potentially improve the outcome of patients with advanced pancreatic tumours safely (29). Additionally, Clustered Regularly Interspaced Short Palindromic Repeats (CRISPR) genetic techniques are being used to ensure precise integration of the genetic material into target cell genomes (17). For example, caspase-9/human FK506-binding protein-hybrids are currently being tested to find out if they promote CAR T-cell apoptosis in response to synthetic molecules that are administered to patients after tumour eradication (17). Suicide genes achieve irreversible depletion of CAR T-cells through the integration of genes like inducible caspase-9 (iCasp9) enzyme (15). These are crucial developments that help improve the safety of CAR T-cell therapy.
Challenges for CAR T-cell therapy in cancer
Despite the benefits, there are challenges with CAR T-cell therapies that can render them less effective. In contrast to physiological T-cell responses, CAR T-cell expansion ex vivo involves exposure to elements that are not encountered by T-cells during in vivo expansion (30). These include viral exposure during transduction, CD3 and CD28 stimulation, and further expansion of recombinant cytokines (30). Also, the expansion of CAR T-cells in vivo is stimulated by lymphopenia and the availability of TAAs (30). The availability of tumour antigens can increase the magnitude of expansion and persistence of CAR T-cells (31).
Antigen escape is an emerging threat to CAR T-cell therapy. One mechanism of antigen escape is the presence of non-functional CD19, which results from deleterious mutations and post-translational modifications (15). The non-functional CD19 cannot be recognized by anti-CD19 CAR T-cells. In 2017, Wang et al. showed that when patients display CD19 isoform that does not possess exon 2, CD19 is no longer able to bind to anti-CD19 CAR T-cells (15). In another mechanism, the leukaemic lineage switch allows immune escape from targeted immunotherapy. For example, two cases of mixed lineage leukaemia (MLL)+ acute myeloid leukaemia (AML) were identified to occur after CD19 CAR T-cell therapy for MLL+ B-acute lymphoblastic leukaemia (ALL) (15, 32). The presence of a stressor (therapy) is likely to select against the leukaemic clone that is found at the time of diagnosis and result in the evolution of a sub-clone that possesses a different phenotype (32). Thus, a lineage switch from CD19+ to CD19- is likely to result in resistance to CAR T-cell therapy. Moreover, the growth of pre-existing rare malignant cells can lead to antigen escape. In 2016, Ruella et al. described that rare CD19-negative CD123-positive cells emerged in great numbers after anti-CD19 CAR T-cell administration (33). These mechanisms highlight the need to develop strategies to prevent and treat antigen escape to enhance the efficacy of CAR T-cell therapy.
CAR T-cell therapy is associated with life-threatening side effects and toxicities. On-target on-tumour toxicity, observed in the treatment of leukaemia and solid tumours, can be caused by rapid destruction of large tumour mass, resulting in the massive release of tumour cell components into the circulation (18). On-target on-tumour toxicity, the most life-threatening toxicity for CAR T-cell therapy, relates to adverse side effects such as cytokine-release syndrome (CRS) (34). CRS was one frequent side effect that was observed in patients who received treatment for haematological malignancies like ALL (18, 35). CRS refers to a non-antigen-specific increased release of pro-inflammatory cytokines due to immune activation (14, 36). Patients present with clinical features like fatigue, high-grade fever, nausea, and cardiac dysfunction (36). While corticosteroids can provide symptomatic relief from the symptoms of inflammation, studies have shown that the use of corticosteroids can cause ablation of the infused CAR T-cells, rendering them ineffective (35). Currently, a more selective IL-6 receptor blocking antibody, tocilizumab, is being used (35, 36). Patients using CAR T-cell therapy can also develop neurological complications like delirium, confusion, and seizure-like activity (35). Though the exact mechanism for neurotoxicity is not defined, the postulated mechanism for neurotoxicity includes diffusion of cytokines and translocation of activated CAR T-cells across the blood-brain barrier (14). Neurotoxicity effects can result independent of CAR T-cell specificity and the type of cancer (18). Patients who are found to have neurological deficits are evaluated with electroencephalograms (EEGs) (to confirm seizure-like activity) and lumbar punctures (to confirm lymphocytosis) (35). Moreover, tumour lysis syndrome (TLS) can also result in patients using CAR T-cell therapy. TLS refers to the rapid death of tumour cells that subsequently alters electrolyte balance in plasma (14). Specifically, metabolic disturbances like hyperuricemia and hyperkalaemia can result from TLS (14). Another type of toxicity, called on-target off-tumour toxicity, is observed in the treatment of solid tumours, leukaemia, and lymphoma (18). On-target off-tumour toxicity originates from the binding of CAR T-cell to target antigens that are also present on the surface of normal cells, leading to the destruction of healthy cells and organs (34, 37).
Future Work
While traditional CAR T-cells detect extracellular antigens, these form only 1% of total cellular proteins which suggests that not a huge number of TAAs are available for detection (38). Compared to CAR T-cell therapy, T-cell receptor engineered T-cells (TCR-T cells) detect intracellular antigens more sensitively via the MHC system (15, 39). Clinical studies using TCR-T cells have achieved significant success in the treatment of myeloma, metastatic melanoma, and colorectal cancer (40, 41, 42). Perhaps, further research on a combination of CAR T-cells and TCR-T cells may help to increase the diversity of TAAs detected.
The success of CAR T-cell therapy has also increased interest in exploring the clinical utility of other immune cell types, such as NK cells. NK cells can recognise tumour cells using a combination of stimulatory and inhibitory receptors, which detect ligands associated with oncogenic transformation (43). Cytotoxicity and targeted cell killing are characteristics of NK cells that can be used in cancer treatment (43). Similar to CAR T-cell therapy, the binding of CAR-expressing NK cells to specific tumour antigens can induce NK cell-mediated cytotoxicity (44). CAR-expressing NK cells have advantages over CAR T-cell therapy. As NK cells are short-lived, there is a reduced risk of autoimmunity or malignant transformation (44). Furthermore, cytokine production by NK cells is considered safer than the cytokine-release syndrome associated with CAR T-cell therapy (44). However, there are challenges associated with manufacturing CAR-expressing NK cells, which include mass production of NK cells from peripheral blood and low transfection efficiency (45). CAR-expressing NK cells are in the early stages of development and further research is underway to determine their safety and efficacy.
Conclusion
While CAR T-cell therapy has shown promising results in the management of B-cell malignancies, further research is necessary to capitalise on its benefits for use in other malignancies like solid tumours. Due to the adverse effects of CAR T-cell therapy, which includes CRS and neurotoxicity, appropriate risk assessment and mitigation strategies need to be developed before clinical use. Research into CAR T-cell therapy has also encouraged studies exploring the role of other immune cells in cancer treatment. The potential of CAR T-cell therapy to treat cancer is indeed an eye-opener to use similar technology for other conditions such as infectious diseases. At the same time, antigen-recognising CARs can be used to protect graft functions in organ transplantations and reduce the recognition of “self” particles in autoimmune conditions.
References
- Hanahan D, Weinberg R. Hallmarks of Cancer: The Next Generation. Cell. 2011;144(5):646-674.
- Ehrlich P. Über den jetzigen Stand der Chemotherapie. Berichte der deutschen chemischen Gesellschaft. 1909;42(1):17-47.
- H Sherwood L. Cellular and Humoral Aspects of the Hypersensitive States: A Symposium at the New York Academy of Medicine. Journal of the American Medical Association. 1959;170(7):883.
- Burnet F. Immunological Surveillance in Neoplasia. Immunological Reviews. 1971;7(1):3-25.
- Vinay D, Ryan E, Pawelec G, Talib W, Stagg J, Elkord E et al. Immune evasion in cancer: Mechanistic basis and therapeutic strategies. Seminars in Cancer Biology. 2015;35:S185-S198.
- Davila M, Sadelain M. Biology and clinical application of CAR T cells for B cell malignancies. International Journal of Hematology. 2016;104(1):6-17.
- Riches J, Davies J, McClanahan F, Fatah R, Iqbal S, Agrawal S et al. T cells from CLL patients exhibit features of T-cell exhaustion but retain capacity for cytokine production. Blood. 2013;121(9):1612-1621.
- Sukari A, Abdallah N, Nagasaka M. Unleash the power of the mighty T cells-basis of adoptive cellular therapy. Critical Reviews in Oncology/Hematology. 2019;136:1-12.
- Ruella M, Kenderian S. Next-Generation Chimeric Antigen Receptor T-Cell Therapy: Going off the Shelf. BioDrugs. 2017;31(6):473-481.
- Tisagenlecleucel for treating relapsed or refractory B-cell acute lymphoblastic leukaemia in people aged up to 25 years. NICE [Internet]. 2021 [cited 10 April 2021]. Available from: https://www.nice.org.uk/guidance/ta554/chapter/1-Recommendations
- Axicabtagene ciloleucel for treating diffuse large B-cell lymphoma and primary mediastinal large B-cell lymphoma after 2 or more systemic therapies. NICE [Internet]. 2021 [cited 10 April 2021]. Available from: https://www.nice.org.uk/guidance/ta559/chapter/1-Recommendations
- Zhang C, Durer S, Thandra KC, Kasi A. Chimeric Antigen Receptor T-Cell Therapy. [Updated 2021 Mar 19]. In: StatPearls [Internet]. Treasure Island (FL): StatPearls Publishing; 2021 Jan. Available from: https://www.ncbi.nlm.nih.gov/books/NBK537294/
- Kershaw M, Westwood J, Parker L, Wang G, Eshhar Z, Mavroukakis S et al. A Phase I Study on Adoptive Immunotherapy Using Gene-Modified T Cells for Ovarian Cancer. Clinical Cancer Research. 2006;12(20):6106-6115.
- Hartmann J, Schüßler‐Lenz M, Bondanza A, Buchholz C. Clinical development of CAR T cells—challenges and opportunities in translating innovative treatment concepts. EMBO Molecular Medicine. 2017;9(9):1183-1197.
- Wang Z, Wu Z, Liu Y, Han W. New development in CAR-T cell therapy. Journal of Hematology & Oncology. 2017;10(1).
- Xia A, Wang X, Lu Y, Lu X, Sun B. Chimeric-antigen receptor T (CAR-T) cell therapy for solid tumors: challenges and opportunities. Oncotarget. 2017;8(52):90521-90531.
- Boyiadzis M, Dhodapkar M, Brentjens R, Kochenderfer J, Neelapu S, Maus M et al. Chimeric antigen receptor (CAR) T therapies for the treatment of hematologic malignancies: clinical perspective and significance. Journal for Immunotherapy of Cancer. 2018;6(1).
- Miliotou A, Papadopoulou L. CAR T-cell Therapy: A New Era in Cancer Immunotherapy. Current Pharmaceutical Biotechnology. 2018;19(1):5-18.
- Akce M, Zaidi M, Waller E, El-Rayes B, Lesinski G. The Potential of CAR T Cell Therapy in Pancreatic Cancer. Frontiers in Immunology. 2018;9.
- Zuo B, Yan B, Zheng G, Xi W, Zhang X, Yang A et al. Targeting and suppression of HER3-positive breast cancer by T lymphocytes expressing a heregulin chimeric antigen receptor. Cancer Immunology, Immunotherapy. 2017;67(3):393-401.
- Zhang Z, Jiang J, Wu X, Zhang M, Luo D, Zhang R et al. Chimeric antigen receptor T cell targeting EGFRvIII for metastatic lung cancer therapy. Frontiers of Medicine. 2019;13(1):57-68.
- Sureban S, Berahovich R, Zhou H, Xu S, Wu L, Ding K et al. DCLK1 Monoclonal Antibody-Based CAR-T Cells as a Novel Treatment Strategy against Human Colorectal Cancers. Cancers. 2019;12(1):54.
- Slaney C, Kershaw M, Darcy P. Trafficking of T Cells into Tumors. Cancer Research. 2014;74(24):7168-7174.
- Wang G, Lu X, Dey P, Deng P, Wu CC, Jiang S, et al. Targeting YAP-dependent MDSC infiltration impairs tumor progression. Cancer Discovery. 2016;6(1):80-95.
- Sermer D, Brentjens R. CAR T‐cell therapy: Full speed ahead. Hematological Oncology. 2019;37(S1):95-100.
- Hegde M, Corder A, Chow K, Mukherjee M, Ashoori A, Kew Y et al. Combinational Targeting Offsets Antigen Escape and Enhances Effector Functions of Adoptively Transferred T Cells in Glioblastoma. Molecular Therapy. 2013;21(11):2087-2101.
- Zah E, Lin M, Silva-Benedict A, Jensen M, Chen Y. T Cells Expressing CD19/CD20 Bispecific Chimeric Antigen Receptors Prevent Antigen Escape by Malignant B Cells. Cancer Immunology Research. 2016;4(6):498-508.
- Gill S, June C. Going viral: chimeric antigen receptor T-cell therapy for hematological malignancies. Immunological Reviews. 2014;263(1):68-89.
- Raj D, Yang M, Rodgers D, Hampton E, Begum J, Mustafa A et al. Switchable CAR-T cells mediate remission in metastatic pancreatic ductal adenocarcinoma. Gut. 2018;68(6):1052-1064.
- McLellan A, Ali Hosseini Rad S. Chimeric antigen receptor T cell persistence and memory cell formation. Immunology & Cell Biology. 2019;97(7):664-674.
- Turtle C, Hanafi L, Berger C, Gooley T, Cherian S, Hudecek M et al. CD19 CAR–T cells of defined CD4+:CD8+ composition in adult B cell ALL patients. Journal of Clinical Investigation. 2016;126(6):2123-2138.
- Perna F, Sadelain M. Myeloid leukemia switch as immune escape from CD19 chimeric antigen receptor (CAR) therapy. Translational Cancer Research. 2016;5(S2):S221-S225.
- Ruella M, Barrett D, Kenderian S, Shestova O, Hofmann T, Perazzelli J et al. Dual CD19 and CD123 targeting prevents antigen-loss relapses after CD19-directed immunotherapies. Journal of Clinical Investigation. 2016;126(10):3814-3826.
- Ma S, Li X, Wang X, Cheng L, Li Z, Zhang C et al. Current Progress in CAR-T Cell Therapy for Solid Tumors. International Journal of Biological Sciences. 2019;15(12):2548-2560.
- Davila M, Riviere I, Wang X, Bartido S, Park J, Curran K et al. Efficacy and Toxicity Management of 19-28z CAR T Cell Therapy in B Cell Acute Lymphoblastic Leukemia. Science Translational Medicine. 2014;6(224):224ra25-224ra25.
- Lee DW, Gardner R, Porter DL, et al. Current concepts in the diagnosis and management of cytokine release syndrome. Blood. 2014;124(2):188-195.
- Morgan R, Yang J, Kitano M, Dudley M, Laurencot C, Rosenberg S. Case Report of a Serious Adverse Event Following the Administration of T Cells Transduced With a Chimeric Antigen Receptor Recognizing ERBB2. Molecular Therapy. 2010;18(4):843-851.
- Zheng Y, Qin L, Zacarías N, de Vries H, Han G, Gustavsson M et al. Structure of CC chemokine receptor 2 with orthosteric and allosteric antagonists. Nature. 2016;540(7633):458-461.
- Harris D, Kranz D. Adoptive T Cell Therapies: A Comparison of T Cell Receptors and Chimeric Antigen Receptors. Trends in Pharmacological Sciences. 2016;37(3):220-230.
- Rapoport A, Stadtmauer E, Binder-Scholl G, Goloubeva O, Vogl D, Lacey S et al. NY-ESO-1–specific TCR–engineered T cells mediate sustained antigen-specific antitumor effects in myeloma. Nature Medicine. 2015;21(8):914-921.
- Parkhurst M, Yang J, Langan R, Dudley M, Nathan D, Feldman S et al. T Cells Targeting Carcinoembryonic Antigen Can Mediate Regression of Metastatic Colorectal Cancer but Induce Severe Transient Colitis. Molecular Therapy. 2011;19(3):620-626.
- Robbins P, Morgan R, Feldman S, Yang J, Sherry R, Dudley M et al. Tumor Regression in Patients With Metastatic Synovial Cell Sarcoma and Melanoma Using Genetically Engineered Lymphocytes Reactive With NY-ESO-1. Journal of Clinical Oncology. 2011;29(7):917-924.
- Chiossone L, Dumas P, Vienne M, Vivier E. Natural killer cells and other innate lymphoid cells in cancer. Nature Reviews Immunology [Internet]. 2018;18(11):671-688.
- Guillerey C, Huntington N, Smyth M. Targeting natural killer cells in cancer immunotherapy. Nature Immunology. 2016;17(9):1025-1036.
- Xia J, Minamino S, Kuwabara K. CAR-expressing NK cells for cancer therapy: a new hope. BioScience Trends. 2020;14(5):354-359.
- Log in to post comments