Endeavouring to Improve Glioblastoma Multiforme Patient Prognosis – A Literature Review of Biomarkers and Novel Therapeutic Approaches
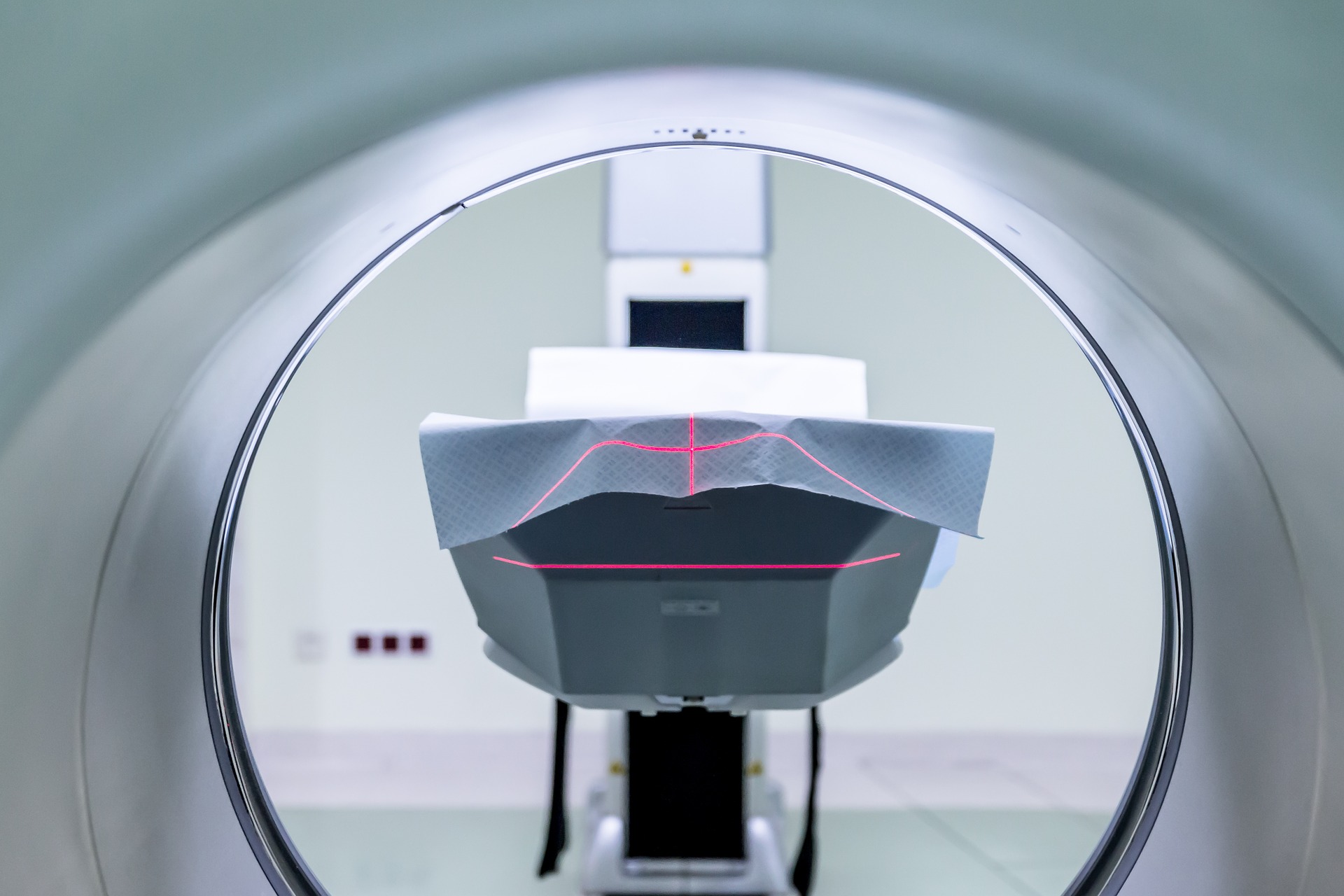
Abstract
Glioblastoma Multiforme (GBM) patients typically deteriorate at a rapid rate, and survive an average of 15 months from diagnosis – despite clinical intervention with the current approach of maximal surgical resection and adjuvant chemoradiotherapy. Tumour recurrence and treatment resistance remain inevitable with this standard treatment protocol, and therefore, we must endeavour to investigate more efficacious alternatives which offer better patients better prognosis and quality of life. GBM is a highly heterogeneous tumour: both between the cells within the tumour itself, and between individual patients. This makes finding a single effective treatment regimen a significant challenge – with a simple, ‘one-size-fits-all’ approach proven to be inadequate. There is therefore great indication to: 1) identify tumour-specific genetic signatures; 2) determine suitable predictive and prognostic biomarkers; 3) develop treatment strategies with specific molecular targets; and 4) develop efficacious agents that have the ability to cross the blood-brain-barrier (BBB) - which impedes the delivery of chemotherapeutics to the tumour site. This review discusses novel therapeutic approaches (e.g. precision medicine, microRNAs, magnetic resonance-guided focused ultrasound (MRgFUS), immunotherapy, nanoparticles) which either improve intratumoral delivery of current chemotherapeutics, or act themselves as a more targeted agent – and therefore, may have the potential to improve the diagnostic burden faced by individuals with this devastating illness.
Introduction
Glioblastoma Multiforme (GBM) is the most common primary brain tumour in adults and is associated with the poorest prognosis. As a particularly aggressive and invasive malignancy of the glial cells of the central nervous system, GBM is classified as a WHO grade IV glioma [1]. Despite maximal surgical resection followed by radiotherapy and adjuvant chemotherapy [2–8], the median survival of GBM patients remains at ~15 months, with less than 5% of cases surviving 5 years from diagnosis [2,3,8,9]. As tumour recurrence and treatment resistance remain inevitable despite current treatment protocol [6], there is a great incentive to improve the delivery and efficacy of existing therapeutics, and to identify new molecular targets.
GBM is very locally invasive, and therefore cannot be completely ‘cured’ by surgical resection, as some infiltrating cells will have already spread beyond the visible tumour margins before surgery [3,7]. Relapse within 2-3cm from the margin of the original lesion occurs in ~80% of cases. Resection is also not possible if the tumour resides in eloquent brain tissue, the brain stem or basal ganglia [10]. Radiotherapy has shown to improve life expectancy in high-grade gliomas. It induces DNA damage to any residual cells following resection, however its cytotoxicity is not tumour-specific - causing radiation-induced necrosis of the normal parenchyma, and neuronal damage [2,7,11]. It is also possible for tumours to become radio-resistant [10].
Current chemotherapeutics used in GBM typically fail - due to the aggressive nature of the tumour; intratumoral and intertumoral heterogenicity; alterations within both tumour cells and other non-malignant cells within the tumour microenvironment (TME) which contribute to resistance; and poor drug delivery across the blood-brain barrier (BBB) [2,12]. Temozolomide (TMZ) is the first-line chemotherapeutic agent used in GBM [2,9,13]. In addition to surgery and radiotherapy, TMZ has shown to increase overall survival in newly diagnosed patients (12.1 to 14.6months) [11]. Intratumoral TMZ delivery remains challenging due to its short half-life; with peak brain serum levels only 17%–20% of that reached in the systemic circulation. Additionally, the obstructive peritumoral BBB explains concentrations decreasing 40-fold from the tumour centre to the peritumour tissue [4,12,13].
Pathogenesis of Glioblastoma Multiforme
GBMs are heterogeneous in macroscopic and histological appearance, and genetic/epigenetic signatures. Macroscopically, GBMs are characterised by multi-focal haemorrhage, necrosis, as well as cystic and gelatinous areas. Histologically, GBMs range from small, poorly differentiated tumour cells to large multi-nucleated cells with necrosis, pseudo-palisading nuclei and frequent mitotic activity [10]. Despite their diversity, all GBMs easily invade the parenchyma but rarely metastasise outside the brain.
In response to detecting changes in the tumour microenvironment (TME), GBM cells adapt in attempt to maintain rapid tumour growth [14]. Examples of pro-tumorigenic genetic adaptations that occur during the pathogenesis of GBM, and are attractive therapeutic targets, are illustrated in Figure 1. Precision medicine can utilise such targets and tailor novel treatments to a patient’s individual genetic profile e.g. EGFR overexpression.
Areas of local hypoxia arise within the TME as the tumour outgrows its blood supply [15]. This triggers neural and/or non-neural cells to release factors which promote angiogenesis and alternative metabolic pathways [15–17] (see figure 1.1). Vascular endothelial growth factor (VEGF) promotes abnormal angiogenesis, and thus has the ability to restore oxygen supply to hypoxic regions of the tumour [18]. As this mechanism is essential for the survival of GBMs, blocking the VEGF pathway and inhibiting angiogenesis is an attractive therapeutic strategy.
Bevacizumab, an anti-VEGF monoclonal antibody, is the most studied anti-angiogenic agent for GBM. Current evidence does not support bevacizumab as a first-line adjuvant treatment for GBM but it may improve survival when combined with chemotherapy [18]. So far, anti-VEGF therapeutics have shown limited efficacy in GBM – due to impaired delivery across the BBB, and inevitable resistance. Despite VEGF-induced neo-vessels having a dysfunctional BBB (due to their large fenestrations and defective tight junction proteins) [2,16], the BBB remains intact within peri-tumour areas, where invading cells are interspersed by healthy brain cells. The peri-tumoral vasculature is therefore a barrier for most therapeutics [19]. Tumour cells can also upregulate alternative signalling pathways in response to VEGF-signalling blockade.
Platelet-derived growth factor (PDGF) ligands (-A, -B and -C) bind to their cognate receptor (PDGFR) to activate signal transduction cascades which promote GBM proliferation and survival. Multiple studies have observed PDGF overexpression in GBM, particularly PDGF-A and -B. PDGF overexpression can occur in response to VEGF-blockade. VEGF and PDGF inhibitors used as a combination therapy, as well as non-specific tyrosine kinase inhibitors (TKIs) (which inhibit both VEGF and PDGF pathways as a single agent) show promise in GBM therapy [1].
Epidermal growth factor (EGF) is secreted in response to TME disruption by the infiltrating tumour and from tumour cells themselves. Upon binding to its corresponding receptor tyrosine kinase (EGFR), EGF re-programmes tumour cell metabolism, allowing proliferation to continue even in hypoxic regions [17]. As EGF promotes further tumour growth, there is further TME disruption, resulting in a vicious cycle [17]. These features explain the common observation of EGFR overexpression in primary GBM (40%) [1] – which are typically more rapidly progressive and invasive than secondary GBMs. EGFR-TKIs shown promise as a targeted therapy for GBM, however these agents need to be improved further, particularly in terms of drug delivery and efficacy [1]. EGFR-type-III (EGFRvIII) is the most common activating EGFR mutation in GBM [14,20]. Anti-EGFR monoclonal antibodies, chimeric antigen receptors (CAR) engineered to recognise EGFRvIII (CAR T-cell therapy), and Rindopepimut vaccination (a peptide targeting EGFRvIII-positive cells), have all been trialled for GBM [14]. Due to these agents failing to penetrate the BBB and heterogenous EGFR expression, EGFR has lost attraction as a therapeutic target for GBM. Instead, recent studies have utilised EGFR as a docking molecule, enabling the transport of effector agents, e.g. nanoparticles (section 8) [21].
The PI3K/AKT/mTOR-pathway induces cell survival and proliferation in response to external cues such as: growth factors, stress, energy status, oxygen and amino acid concentration. Phosphatidylinositol 3-kinase (PI3K) activates serine/threonine-specific protein kinase (AKT) through phosphorylation. Subsequently, AKT phosphorylates and activates the mammalian target of rapamycin (mTOR). PTEN (phosphatase and tensin homolog), a tumour suppressor which antagonizes PI3K signaling, negatively regulates the PI3K/AKT/mTOR-pathway (see figure 1.2). GBMs with PTEN deletion, and therefore overactivation of the PI3K/AKT/mTOR pathway, have generally shorter survival than those with PTEN retention [22].
Isocitrate dehydrogenase (IDH) is an enzyme with a key role in the citric acid cycle. It exists in three isoforms: IDH1, which is found in peroxisomes and cytoplasm, and IDH2 and 3, which both reside within the mitochondria. Through oxidative decarboxylation, wildtype IDH1/2 convert isocitrate and NADP+ into a-ketoglutarate (a-KG), NADPH and carbon dioxide. -KG is an essential co-factor for several enzymes [1]. IDH1/2 gene mutations, and subsequently altered/mutant IDH1/2 enzymes, are present in some subtypes of GBM. These mutant enzymes exert their pro-oncogenic action through their association with altered hypoxia sensing, and increased histone and DNA methylation [23]. The mutant IDH1/2 enzymes have decreased binding affinity for isocitrate, preventing its conversion to -KG, and increased binding affinity for NADPH. This results in an incomplete reaction by only reducing -KG (without carboxylation), resulting in the formation of 2-hydroxyglutarate (2-HG) (see figure 1.3). 2-HG is considered to be an oncometabolite, and is responsible for oncogenic transformation [1].
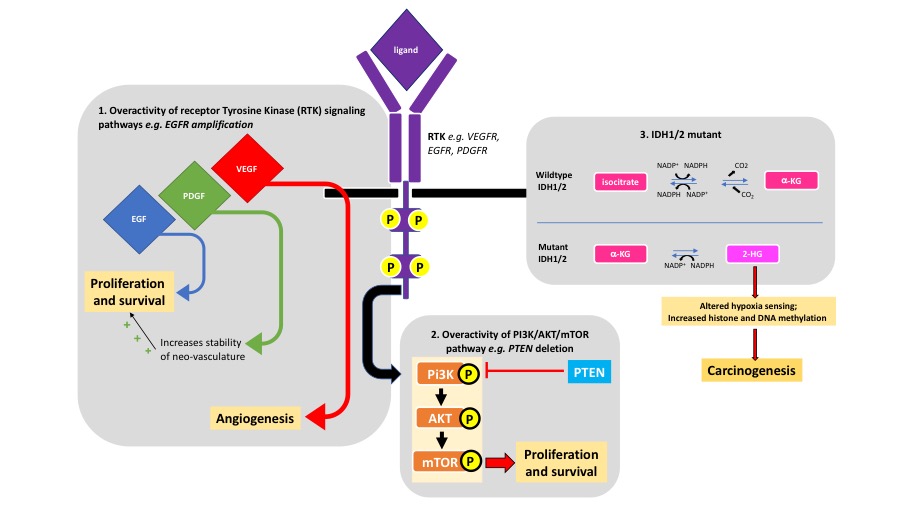
Figure 1: Molecular Features and Adaptations Involved in the Pathogenesis of Glioblastoma Multiforme
Abbreviations: EGF = epidermal growth factor; EGFR = EGF receptor; PDGF = platelet derived growth factor; PDGFR = PDGF receptor; VEGF = vascular endothelial growth factor; VEGFR = VEGF receptor; RTK = receptor tyrosine kinase; PI3K = phosphatidylinositol 3-kinase; AKT = serine/threonine-specific protein kinase; mTOR = mammalian target of rapamycin; PTEN = phosphatase and tensin homolog; IDH = isocitrate dehydrogenase; -KG = -ketoglutarate; 2-HG = 2-hydroxyglutarate. The above molecular features and adaptations can be used to determine the specific ‘genetic profile’ of GBMs, as well as deciphering predictive and prognostic biomarkers. Additionally, precision medicine can utilise specific molecular targets, such as those illustrated above, to tailor novel treatments to a patient’s individual genetic profile. 1. RTK signalling pathways are often hyperactive in GBM. This can occur in response to conditions within the TME, or, alternative signalling pathways can be upregulated as a mechanism of treatment resistance to inhibition of a specific RTK. EGFR overexpression in primary GBM (40%) (1) – which are typically more rapidly progressive and invasive than secondary GBMs; 2. GBMs with PTEN deletion, and therefore overactivation of the PI3K/AKT/mTOR pathway, have generally shorter survival than those with PTEN retention (22); 3. IDH1/2 mutations are more common in secondary GBM, which is typically associated with better prognosis.
Genetic heterogeneity between both GBM patients and the cells which comprise a single tumour makes identifying these targets a challenge. One of many groups searching for novel therapeutic targets for GBM, Larsson et al. (2020), highlighted novel targets must be essential for gliomagenesis, but their inhibition must prove non-toxic to healthy brain tissue [9].
GBM Subtyping & Prognostic and Predictive Biomarkers
IDH1/2 Mutation Status
GBM can be divided into 3 subtypes based on IDH1/2 mutation status – IDH-mutant, IDH-wildtype and NOS (not otherwise specified) (see table 1). Subtyping GBM by IDH1/2 mutation status may help in both diagnosis, and the designing of targeted treatment strategies.
Primary GBMs account for the vast majority of all GBM cases. They are typically IDH-wildtype, and develop de novo – presenting in older patients and associated with a shorter clinical history or rapid worsening of symptoms. The acquisition of multiple genetic alterations results in the early and rapid progression of these tumours into WHO grade IV GBMs [22]. These GBMs feature a more aggressive infiltration pattern and poorer prognosis [8,11,14,16]. Alterations which are considered ‘hallmarks’ of primary GBM include: EGFR mutation and amplification, MDM2 overexpression, and LOH of chromosome 10q - holding PTEN and TERT-promoter mutations [7].
Secondary GBMs, characterized by IDH-mutations, gradually develop from previously diagnosed WHO grade II or grade III gliomas. Secondary GBM are less common, tend to occur in younger patients, and are associated with a more favourable outcome [7,11,12,18]. IDH1/2 mutations can be followed by 1p/19q co-deletion or co-operating TP53, ATRX or TERT-promoter mutations [10]. IDH-mutant GBMs with secondary T53 and/or ATRX mutations progress from tumours of an astrocytic lineage, whereas GBMs with loss of 1p/19q and TERT-promoter mutation develop from oligodendrogliomas [16,23]. TERT promotor mutations occur in the highest incidence among tumours harboring 1p/19q co-deletion, as well as in those with ATRX mutations [22].
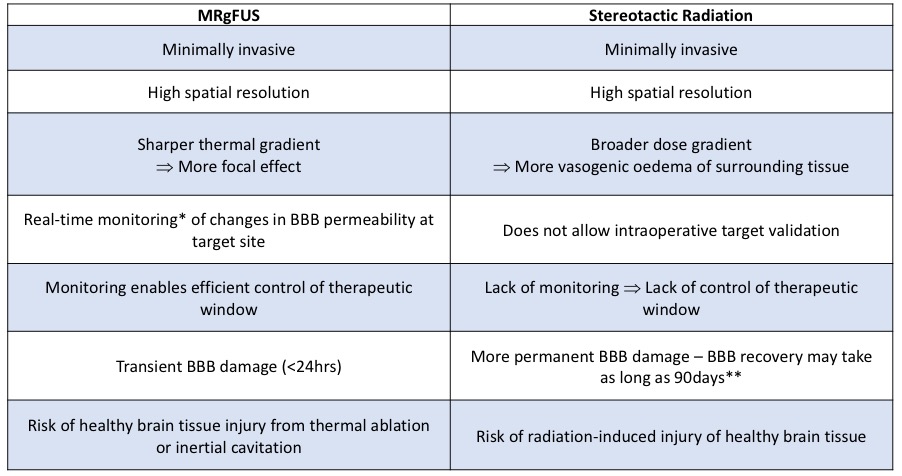
Table 1: Primary and Secondary GBM – Subtyping Based on IDH-Mutation Status
(Abbreviations: IDH = isocitrate dehydrogenase; GBM = glioblastoma multiforme; OS = overall survival; TERT = telomerase reverse transcriptase; EGFR = epidermal growth factor receptor; PTEN = phosphatase and tensin homolog; TP53 = tumour protein p53; MDM2 = mouse double minute 2; ATRX = a-thalassaemia/mental retardation syndrome X-linked; LOH = loss of heterozygosity). IDH1 mutations are commonly established as a hallmark molecular feature of secondary GBM (70%). Generally, IDH mutations are less frequent in primary GBM (only 5-20% of primary GBMs are IDH1-mutant)[22]. IDH-mutation followed by T53 mutation and/or ATRX mutation is associated with progression of tumours of an astrocytic lineage, whereas IDH-mutation followed by 1p/19q co-deletion along with TERT promoter mutation is associated with oligodendrogliomas. TERT promotor mutations occur in IDH-wildtype with EGFR-amplification. TERT promotor mutations occur in the highest incidence among tumours harboring 1p/19q co-deletion, as well as in those with ATRX mutations [22]. PTEN deletions are fairly common across all gene expression subtypes of GBM, but are absent in IDH1 mutant tumours. GBMs featuring LOF mutations of PTEN have been found to generally have shorter survival than those with PTEN retention [22].
Mutant IDH (mIDH) inhibitors have been investigated as a novel targeted cancer therapy, with the ability to block mIDH to produce 2-HG and induce expression of genes involved in promoting gliomagenesis. An on-going phase I trial of AG-221 (a selective mIDH2 inhibitor) and AG881 (a non-specific IDH inhibitor) aims to further understand the ability of these agents to reduce 2-HG levels, by comparing concentrations in resected and treated tumours from IDH1 mutant glioma patients to the 2-HG concentration of the untreated tumour [1].
Gene Expression-Based Molecular Classification of GBM
Data from The Cancer Genome Atlas has further enabled the molecular pathogenesis and gene expression-based molecular classification of GBMs into: classical, mesenchymal, pro-neural and neural subtypes. Classical GBM is characterised by large-scale amplification of EGFR, as well as loss of PTEN and CDKN2A. Pro-neural GBM is most commonly characterised by mutations in IDH1 and PDGFR-A. Mesenchymal GBM is typically associated with mutations in neurofibromin 1 (NF1) [10,23]. No unique genetic signature has been determined for the neural subtype [10]. Further identification of characteristic and highly frequent molecular alterations will aid GBM classification and guide the improvement of therapeutic strategies and development of novel therapeutics for this ‘incurable’ malignancy.
CpG Island Methylator Phenotype
IDH1/2 mutant GBMs typically feature a CpG island methylator phenotype (G-CIMP). G-CIMP-positive GBMs have been linked with IDH1 mutations and correlate with better prognosis [23]. Methylation of the promotor region of the gene encoding MGMT (06-methylguanine-DNA methyltransferase), may be involved with this G-CIMP phenomenon – as it is found in almost all G-CIMP positive GBMs (see figure 2).
MGMT Methylation
MGMT, a DNA-repair enzyme which removes alkyl groups from guanine bases, antagonises the alkylating action of temozolomide (TMZ),[7,12]. MGMT promoter methylation, which silences the MGMT gene, prevents MGMT-mediated DNA repair and tumour cell survival. Loss of MGMT-mediated DNA repair explains the association of MGMT methylation with better response to TMZ and longer overall survival [5]. Conversely, unmethylated MGMT promoters are associated with poorer prognosis [7]. As a predictive biomarker, MGMT methylation is particularly important for guiding adjuvant therapy in elderly GBM patients. Its use helps to determines the best therapy for these patients aiding in the decision to include TMZ – stratifying patients who should be treated with radiotherapy only (MGMT unmethylated), and patients who benefit more from TMZ alone or combined TMZ/radiotherapy (MGMT methylated) (see figure 2) [23].
MicroRNAs
microRNAs (miRNAs) are non-coding RNA molecules of ~22 nucleotides in length. Following interaction with their target mRNA, they cause mRNA degradation or prevent protein translation. Target protein function determines if the up-/down-regulated miRNA is tumour-suppressive or oncogenic [7]. The significance of specific miRNAs as potential GBM therapeutic targets, or biomarkers, remains uncertain [7,16].
MGMT expression does not always correlate with promoter status (e.g. unmethylated MGMT patients with comparable long-term survival) and could be explained by specific miRNAs influencing MGMT expression. Kirstein et. al (2020) found eight miRNAs to regulate MGMT expression, with five increasing tumour sensitivity to alkylating agents [7]. Therefore, targeting miRNAs could be a potential personalised treatment strategy for MGMT-unmethylated patients, by reducing MGMT overexpression and TMZ resistance.
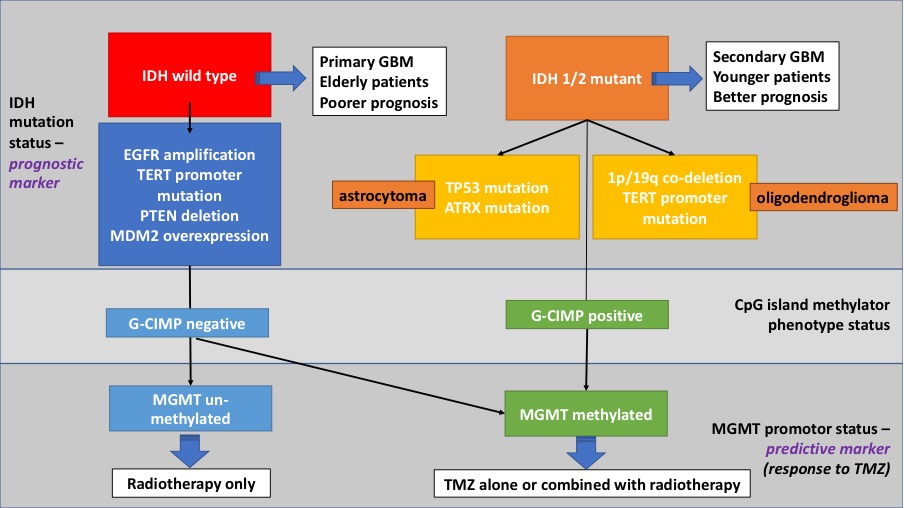
Figure 2: Clinical Applications of IDH mutation, CpG island methylator phenotype and MGMT promoter statuses
(Abbreviations: IDH = isocitrate dehydrogenase; GBM = glioblastoma multiforme; EGFR = epidermal growth factor receptor; TERT = telomerase reverse transcriptase; PTEN = phosphatase and tensin homolog; MDM2 = mouse double minute 2; TP53 = tumour protein P53; ATRX = a-thalassaemia/mental retardation syndrome X-linked; G-CIMP = CpG island methylator phenotype; MGMT = 06-methylguanine-DNA methyltransferase; TMZ = temozolomide) IDH1/2 mutation status aids the sub-classification of primary and secondary GBM. This enables the determination of tumours with worse and better prognosis, respectively. IDH1/2 mutant GBMs typically feature a CpG island methylator phenotype (G-CIMP). G-CIMP positive GBMs have been linked with IDH1 mutations and correlate with better prognosis [23]. MGMT methylation may be involved with this G-CIMP phenomenon – as it is found in almost all G-CIMP positive GBMs, but in addition, is present in a subset of G-CIMP negative GBMs. MGMT promotor methylation status can be used as a predictive biomarker in the response of GBM tumours to TMZ chemotherapy. As a predictive biomarker, MGMT methylation is particularly important for guiding adjuvant therapy in elderly GBM patients, who are typically IDH wild type (associated with older age at diagnosis). MGMT methylation analysis enables the stratification of patients who should be treated with radiotherapy only (i.e. MGMT unmethylated), and patients who benefit more from TMZ alone or combined TMZ/radiotherapy (i.e. MGMT methylated) [18].
Barriers to GBM Therapies
The blood-brain barrier (BBB) prevents the entry of neurotoxins and, unfortunately, most therapeutics into the brain [11,13]. The BBB is a selective, semi-permeable barrier composed of adjacent capillary endothelial cells which are joined together by tight junctions [11]. Tight junctions are hydrophilic, and ~0.8nm in diameter. They inhibit paracellular transport of 98% of low molecular weight therapeutics (<400Da) and 100% of large molecule therapeutics [2,19]. The endothelial membrane is a hydrophobic, phospholipid bilayer. Features which enable drugs to more easily undergo transcellular passive diffusion across the membrane include: a low molecular weight, neutral charge / partial ionisation, and lipophilicity [11]. Hydrophilic compounds undergo facilitated diffusion along their concentration gradient via solute-specific ATP-dependent transmembrane proteins (receptor-mediated endocytosis). Transport of drugs with ionizable groups is aided by their non-specific interaction with charged membrane surface domains on the BBB (adsorption-mediated transcytosis) [2].
TME neo-vessels, with larger fenestrations and tight junction defects, can be exploited to enhance drug delivery [8]. The EPR effect describes the extravasation of drugs through the TME vasculature, followed by drug retention as a result of poor lymphatic drainage from the tumour interstitium [2,8,13,21,24]. The EPR effect is affected by pH, polarity and molecular size [13]. Molecules <400nm can cross the ‘leaky’ TME vasculature, however, nanoparticles (5–200nm) show better EPR effect (section 6) [8,24].
Intrinsic resistance indicates resistance-mediating factors existed within tumour cells before receiving chemo/radiotherapy. Acquired resistance develops in GBMs initially sensitive to chemo/radiotherapy, and explains relapse in 90% of cases. Acquired resistance can result from mutations, increased therapeutic target expression or activation of compensatory signalling pathways [2]. Examples of such resistance-mediating signalling pathways and tumour cell expression profiles are illustrated in Figure 1. Some ATP-dependent transporters (e.g. P-glycoprotein) expressed on BBB cells control the efflux of chemotherapeutics against their concentration gradient [2,4]. Decreased P-gp expression observed following BBB disruption indicates a role for modalities that induce targeted BBB damage in treatment-resistant GBM [4].
Magnetic Resonance-guided Focused Ultrasound (MRgFUS)
MRgFUS is an incisionless method of destroying target tissue (e.g. tumour cells), whilst sparing adjacent healthy tissue [4]. Individual beams of FUS energy converge at the target to cause heat and/or mechanical stress [13].
Low-intensity FUS in continuous-wave mode generates a gradual, small temperature rise in the target tissue. High-intensity generates a rapid temperature rise, and at >60oC causes protein denaturation and coagulative necrosis (thermal ablation) [19]. FUS in a pulsatile manner causes acoustic cavitation. Upon penetrating the target tissue, pressure waves interact with dissolved gases. The refractive component of the waves generates gas-filled microbubbles (MBs). Low-intensity waves cause MBs to oscillate. At amplitudes 0.9MPa MBs abruptly collapse, generating violent shock-waves that destroy target cells (inertial cavitation) [13,19].
MRgFUS can improve delivery of GBM therapeutics via: 1) transient BBB disruption; 2) sonoporation and drug trapping; and 3) targeted drug release with carrier vehicles [13].
MRgFUS can disrupt the endothelial membrane and/or tight junctions comprising the BBB [13]. MRgFUS-induced BBB disruption may be a plausible method to enable the use of therapeutic agents which were previously dismissed (due to their inability to cross the BBB). However, this BBB disruption must be transient and reversible - in order to prevent accumulation of neurotoxins within the brain.
In patients receiving chemotherapy and MRgFUS, Mainprize et al (2018) observed elevated chemotherapy concentrations within sonicated versus un-sonicated tissue. Gadolinium enhancement of the sonicated tissue resolved within 24hrs, indicating MRgFUS was successful to induce reversible BBB disruption [13]. Additionally, results from a recent single-center, single-arm study (NCT03712293) on six GBM patients receiving standard adjuvant TMZ chemotherapy showed that multiple BBB disruptions using MRgFUS can be regarded as a safe and accurate procedure. The survival rate up to 1 year was 100%, and none of the patients had immediate or delayed BBB disruption-related complications. However, both a larger patient cohort and a longer follow-up period is required to accurately evaluate the efficacy of MRgFUS-induced BBB disruption in increasing TMZ concentration [25].
High-dose radiation can also cause BBB disruption, but the effect is more permanent [13]. Targeted BBB disruption induced by both stereotactic radiation and MRgFUS is compared in Table 2.
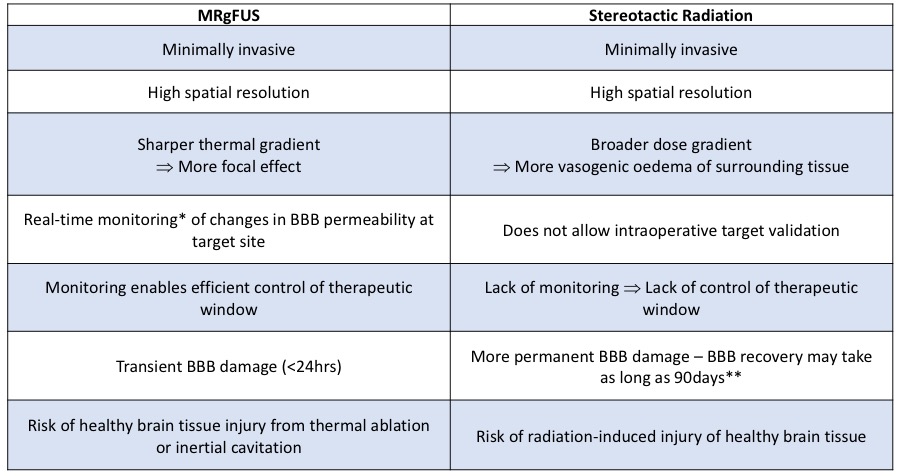
Table 2: Comparison of MRgFUS and Radiation as BBB Opening Methods
(Abbreviations: MRgFUS = magnetic-resonance guided focused ultrasound; BBB = blood-brain-barrier)
*The US machine is bolted within the MRI scanner to allow real time imaging with MR-thermography, which monitors the thermal rise used to create a lesion within the desired location [19,24] **Indicated by the resolution of post-irradiation cerebral oedema 90 days after sonication [4]. Despite the advantages outlined in the table above, there are some disadvantages to using MRgFUS. Patients are required to spend 3-4 hours in the scanner, with head shaved and immobilised in pins. Most importantly, MRgFUS-induced thermal ablation or inertial cavitation can create true intra-cranial lesions – with the risk of permanent effects, e.g. neurological deficit (19).
The difficulty with treating tumours such as GBM is in controlling and monitoring FUS exposure – ensuring adequate tumour necrosis (or in this case, BBB opening) whilst minimizing damage to the normal parenchyma. The therapeutic window of radiation in its use in BBB-opening is also yet to be determined, as it is capable of causing injury of healthy brain tissue and cerebral vasculature, as well as to the BBB endothelial cells [2,4].
Sonoporation following systemic injection of MBs can augment MRgFUS-induced BBB opening [13]. Following stable cavitation of MBs, shock-waves create pores in the endothelial membrane and disrupt tight junctions. This occurs immediately and lasts ~4-6hrs [19]. Intracellular drug trapping occurs once sonoporation terminates [13]. Using MBs also lowers the threshold for inertial cavitation, allowing a lower-intensity MRgFUS to successfully cause BBB-disruption (avoiding healthy tissue damage seen at higher amplitudes) [13,19].
Targeted drug release involves encapsulating the drug in or binding it to a systemically-injected carrier vehicle (e.g. nanoparticles). The therapeutic is then released at the target following a stimulus e.g. temperature or pressure changes following MRgFUS [19].
Nanoparticles
Nanoparticles (NPs) have the potential to improve MRgFUS-induced thermal ablation to the BBB, and target the delivery of drugs specifically to GBM cells [8]. Favourable NP characteristics include: strong affinity for biomolecules specific to GBM cells or the BBB, ability to cross the BBB via receptor-mediated endocytosis, and better EPR effect than larger therapeutics [21].
Affinity molecules (e.g. antibodies, specific receptor molecules) can be conjugated to the NP surface. Those selective for BBB surface molecules enable focused dissipation of thermal energy to the BBB, preventing unwanted damage to surrounding neural cells by MRgFUS [13].
Using NPs as nanocarriers could optimise: BBB transport, half-life, and targeted release of GBM therapies [2,24]. Śamec et. al (2020) proposed NPs should be coated in surfactants to increase their hydrophilicity, as hydrophilic NPs have better BBB penetration [2,24]. PEGylation is the addition of poly(ethylene-glycol) (PEG) chains to the NP surface [2]. The hydrophilic chains interact with water molecules, forming a ‘hydrated’ layer, preventing complement adhesion, opsonisation and subsequent immune clearance or degradation. PEGylation, making NPs more hydrophilic [2], prolongs NP half-life and enhances exposure of the encapsulated therapeutic to the BBB for uptake.
For GBM, designing NPs with tumour-specific affinity molecules could improve the targeted delivery and intratumoral accumulation of TMZ [19,21,24]. Fang et al. (2015) synthesised a tumour-specific TMZ nanocarrier with CTX (a peptide which specifically binds glioma and other malignant cells) conjugated to the NP surface. NP-TMZ-CTX had a 7-fold greater half-life, and between 2- and 6-fold higher uptake than free TMZ. Therefore, NP-TMZ-CTX was able to deliver a more targeted, therapeutic dose of TMZ to GBM cells. This means NP-TMZ-CTX could achieve therapeutic intratumoral TMZ concentrations at smaller systemic doses, reducing the risk of non-specific DNA-damage [12].
Immunotherapy
GBMs feature increased regulatory T-cell recruitment through the ‘leaky’ TME [6,17,24]. Regulatory T-cells are immunosuppressive. Their secretion of TGF-β and IL-10 hinders the anti-tumour function of CD8+ T-cells. CD8+ T-cell concentrations are typically reduced in GBM, with higher concentrations associated with favourable outcome and survival [6,24]. Tumor-associated macrophages (TAMs) comprising ~50% of the TME create a supportive stroma for tumour cell invasion [8]. TAM function and prevalence is determined by GBM-derived factors such as colony-stimulation factor 1 (CSF-1). Both immuno-permissive and immunosuppressive TAMs exist, with the latter being associated with CD8+ T-cell inhibition, through upregulation of TGF-B, arginase and inducible nitric oxide synthase, and poorer patient outcome [6,24].
Immunotherapeutic agents have yet to show success in GBM due to the inability of larger molecules (e.g. antibodies, CAR T-cells) to cross the BBB and access the ‘immune privileged’ brain, and the immunosuppressive nature of GBM itself [6]. MRgFUS and nanocarriers both show promise in improving delivery of immunotherapeutic agents. Novel approaches could also attempt to sensitise GBM to existing immunotherapeutics, e.g. downregulating immunosuppressive TAMs with CSF-1R inhibition, or increasing CD8+ T cell influx into the tumour [6].
Conclusion
Current GBM therapies remain unsuccessful in combatting recurrence or resistance. This indicates an urgency for neuroscientific research to focus on enhancing the treatment options available to patients with this devastating illness, improving both prognosis and quality of life outcomes. The search for new GBM targets must continue in order for personalised therapies against this highly heterogenous tumour to be developed. Studies focusing on uncovering novel GBM targets and the genomic characterisation of single cells within the TME will help combat the issue of both diversity in genetic profiles of patient tumours and between individual tumour cells, as well as identifying and determining the role of non-malignant cell types within the TME - as cell-to-cell interactions between both malignant and non-malignant cells have been discovered to contribute to gliomagenesis and treatment resistance.
Although some GBM targets have been established (e.g. IDH1/2 mutations, MGMT-methylation, VEGF over-expression, immunosuppressive TAM upregulation, CD8+ T-cell downregulation), agents which utilise these targets have yet to demonstrate a substantial benefit in both survival outcomes and tumour progression. Better results may be achieved if previously unsuccessful targeted therapies are trialled in conjunction with novel approaches, such as: targeted delivery with nanocarriers, transient BBB opening with MRgFUS, or strategies which sensitise tumours to immunotherapeutics.
References
[1] Rajaratnam V, Islam MM, Yang M, Slaby R, Ramirez HM, Mirza SP. Glioblastoma: Pathogenesis and Current Status of Chemotherapy and Other Novel Treatments. Cancers (Basel) 2020;12:937. https://doi.org/10.3390/cancers12040937.
[2] Jena L, McErlean E, McCarthy H. Delivery across the blood-brain barrier: nanomedicine for glioblastoma multiforme. Drug Deliv Transl Res 2020;10:304–18. https://doi.org/10.1007/s13346-019-00679-2.
[3] Yool AJ, Ramesh S. Molecular Targets for Combined Therapeutic Strategies to Limit Glioblastoma Cell Migration and Invasion. Front Pharmacol 2020;11:358. https://doi.org/10.3389/fphar.2020.00358.
[4] Mainprize T, Lipsman N, Huang Y, Meng Y, Bethune A, Ironside S, et al. Blood-Brain Barrier Opening in Primary Brain Tumors with Non-invasive MR-Guided Focused Ultrasound: A Clinical Safety and Feasibility Study. Sci Rep 2019;9:321. https://doi.org/10.1038/s41598-018-36340-0.
[5] Feldheim J, Kessler AF, Monoranu CM, Ernestus R-I, Löhr M, Hagemann C. Changes of O(6)-Methylguanine DNA Methyltransferase (MGMT) Promoter Methylation in Glioblastoma Relapse-A Meta-Analysis Type Literature Review. Cancers (Basel) 2019;11:1837. https://doi.org/10.3390/cancers11121837.
[6] Weenink B, French PJ, Sillevis Smitt PAE, Debets R, Geurts M. Immunotherapy in Glioblastoma: Current Shortcomings and Future Perspectives. Cancers (Basel) 2020;12:751. https://doi.org/10.3390/cancers12030751.
[7] Kirstein A, Schmid TE, Combs SE. The Role of miRNA for the Treatment of MGMT Unmethylated Glioblastoma Multiforme. Cancers (Basel) 2020;12:1099. https://doi.org/10.3390/cancers12051099.
[8] Delgado-Martín B, Medina MÁ. Advances in the Knowledge of the Molecular Biology of Glioblastoma and Its Impact in Patient Diagnosis, Stratification, and Treatment. Adv Sci (Weinheim, Baden-Wurttemberg, Ger 2020;7:1902971. https://doi.org/10.1002/advs.201902971.
[9] Larsson I, Uhlén M, Zhang C, Mardinoglu A. Genome-Scale Metabolic Modeling of Glioblastoma Reveals Promising Targets for Drug Development. Front Genet 2020;11:381. https://doi.org/10.3389/fgene.2020.00381.
[10] Hanif F, Muzaffar K, Perveen K, Malhi SM, Simjee SU. Glioblastoma Multiforme: A Review of its Epidemiology and Pathogenesis through Clinical Presentation and Treatment. Asian Pac J Cancer Prev 2017;18:3–9. https://doi.org/10.22034/APJCP.2017.18.1.3.
[11] Taylor OG, Brzozowski JS, Skelding KA. Glioblastoma Multiforme: An Overview of Emerging Therapeutic Targets. Front Oncol 2019;9:963. https://doi.org/10.3389/fonc.2019.00963.
[12] Fang C, Wang K, Stephen ZR, Mu Q, Kievit FM, Chiu DT, et al. Temozolomide Nanoparticles for Targeted Glioblastoma Therapy. ACS Appl Mater Interfaces 2015;7:6674–82. https://doi.org/10.1021/am5092165.
[13] Appelboom G, Detappe A, LoPresti M, Kunjachan S, Mitrasinovic S, Goldman S, et al. Stereotactic modulation of blood-brain barrier permeability to enhance drug delivery. Neuro Oncol 2016;18:1601–9. https://doi.org/10.1093/neuonc/now137.
[14] Wirsching H-G, Galanis E, Weller M. Chapter 23 - Glioblastoma. In: Berger MS, Weller MBT-H of CN, editors. Gliomas, vol. 134, Elsevier; 2016, p. 381–97. https://doi.org/https://doi.org/10.1016/B978-0-12-802997-8.00023-2.
[15] Cohen AL, Colman H. Glioma Biology and Molecular Markers BT - Current Understanding and Treatment of Gliomas. In: Raizer J, Parsa A, editors., Cham: Springer International Publishing; 2015, p. 15–30. https://doi.org/10.1007/978-3-319-12048-5_2.
[16] Silantyev AS, Falzone L, Libra M, Gurina OI, Kardashova KS, Nikolouzakis TK, et al. Current and Future Trends on Diagnosis and Prognosis of Glioblastoma: From Molecular Biology to Proteomics. Cells 2019;8:863. https://doi.org/10.3390/cells8080863.
[17] Pudełek M, Król K, Catapano J, Wróbel T, Czyż J, Ryszawy D. Epidermal Growth Factor (EGF) Augments the Invasive Potential of Human Glioblastoma Multiforme Cells via the Activation of Collaborative EGFR/ROS-Dependent Signaling. Int J Mol Sci 2020;21:3605. https://doi.org/10.3390/ijms21103605.
[18] Ameratunga M, Pavlakis N, Wheeler H, Grant R, Simes J, Khasraw M. Anti‐angiogenic therapy for high‐grade glioma. Cochrane Database Syst Rev 2018. https://doi.org/10.1002/14651858.CD008218.pub4.
[19] Palys V. Focused Ultrasound (s. MRgFUS, transcranial MRI-guided Focused US). Viktor’s Notes Neurosurg Resid 2020:Rx15(1-17). http://www.neurosurgeryresident.net/Rx. Treatment Modalities/Rx15. Focused Ultrasound.pdf (accessed August 18, 2020).
[20] Noorani I, de la Rosa J, Choi YH, Strong A, Ponstingl H, Vijayabaskar MS, et al. PiggyBac mutagenesis and exome sequencing identify genetic driver landscapes and potential therapeutic targets of EGFR-mutant gliomas. Genome Biol 2020;21:181. https://doi.org/10.1186/s13059-020-02092-2.
[21] Westphal M, Maire CL, Lamszus K. EGFR as a Target for Glioblastoma Treatment: An Unfulfilled Promise. CNS Drugs 2017;31:723–35. https://doi.org/10.1007/s40263-017-0456-6.
[22] Li Q-J, Cai J-Q, Liu C-Y. Evolving Molecular Genetics of Glioblastoma. Chin Med J (Engl) 2016;129:464–71. https://doi.org/10.4103/0366-6999.176065.
[23] Aldape K, Zadeh G, Mansouri S, Reifenberger G, von Deimling A. Glioblastoma: pathology, molecular mechanisms and markers. Acta Neuropathol 2015;129:829–48. https://doi.org/10.1007/s00401-015-1432-1.
[24] Šamec N, Zottel A, Videtič Paska A, Jovčevska I. Nanomedicine and Immunotherapy: A Step Further towards Precision Medicine for Glioblastoma. Molecules 2020;25:490. https://doi.org/10.3390/molecules25030490.
[25] Park SH, Kim MJ, Jung HH, Chang WS, Choi HS, Rachmilevitch I, et al. One-Year Outcome of Multiple Blood-Brain Barrier Disruptions With Temozolomide for the Treatment of Glioblastoma. Front Oncol 2020;10:1663. https://doi.org/10.3389/fonc.2020.01663.
Image credits: Image by Michal Jarmoluk from Pixabay
- Log in to post comments